Abstract
Medium- and high-entropy alloys (M/HEAs) mix several principal elements with near-equiatomic composition and represent a model-shift strategy for designing previously unknown materials in metallurgy1,2,3,4,5,6,7,8, catalysis9,10,11,12,13,14 and other fields15,16,17,18. One of the core hypotheses of M/HEAs is lattice distortion5,19,20, which has been investigated by different numerical and experimental techniques21,22,23,24,25,26. However, determining the three-dimensional (3D) lattice distortion in M/HEAs remains a challenge. Moreover, the presumed random elemental mixing in M/HEAs has been questioned by X-ray and neutron studies27, atomistic simulations28,29,30, energy dispersive spectroscopy31,32 and electron diffraction33,34, which suggest the existence of local chemical order in M/HEAs. However, direct experimental observation of the 3D local chemical order has been difficult because energy dispersive spectroscopy integrates the composition of atomic columns along the zone axes7,32,34 and diffuse electron reflections may originate from planar defects instead of local chemical order35. Here we determine the 3D atomic positions of M/HEA nanoparticles using atomic electron tomography36 and quantitatively characterize the local lattice distortion, strain tensor, twin boundaries, dislocation cores and chemical short-range order (CSRO). We find that the high-entropy alloys have larger local lattice distortion and more heterogeneous strain than the medium-entropy alloys and that strain is correlated to CSRO. We also observe CSRO-mediated twinning in the medium-entropy alloys, that is, twinning occurs in energetically unfavoured CSRO regions but not in energetically favoured CSRO ones, which represents, to our knowledge, the first experimental observation of correlating local chemical order with structural defects in any material. We expect that this work will not only expand our fundamental understanding of this important class of materials but also provide the foundation for tailoring M/HEA properties through engineering lattice distortion and local chemical order.
This is a preview of subscription content, access via your institution
Access options
Access Nature and 54 other Nature Portfolio journals
Get Nature+, our best-value online-access subscription
$29.99 / 30 days
cancel any time
Subscribe to this journal
Receive 51 print issues and online access
$199.00 per year
only $3.90 per issue
Rent or buy this article
Prices vary by article type
from$1.95
to$39.95
Prices may be subject to local taxes which are calculated during checkout
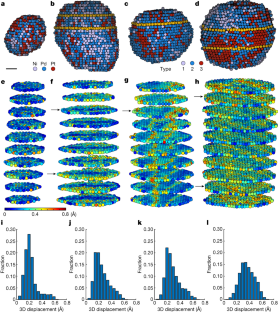
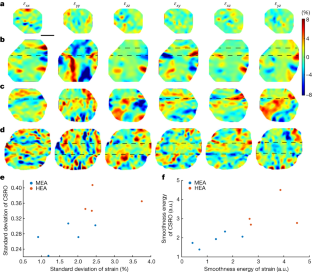
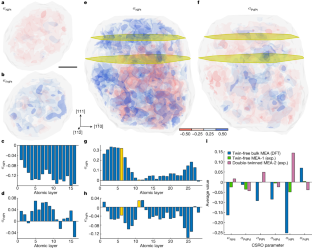
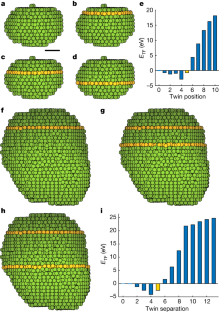
Data availability
All the raw and processed experimental data are available on GitHub (https://github.com/AET-MEA-HEA/Supplementary-Data-Codes).
Code availability
All the MATLAB source codes for the 3D reconstruction, atom tracing and data analysis of this paper are available on GitHub (https://github.com/AET-MEA-HEA/Supplementary-Data-Codes).
References
-
Yeh, J.-W. et al. Nanostructured high-entropy alloys with multiple principal elements: Novel alloy design concepts and outcomes. Adv. Eng. Mater. 6, 299–303 (2004).
-
Cantor, B., Chang, I. T. H., Knight, P. & Vincent, A. J. B. Microstructural development in equiatomic multicomponent alloys. Mater. Sci. Eng. A 375–377, 213–218 (2004).
-
Gludovatz, B. et al. A fracture-resistant high-entropy alloy for cryogenic applications. Science 345, 1153–1158 (2014).
-
Li, Z., Pradeep, K. G., Deng, Y., Raabe, D. & Tasan, C. C. Metastable high-entropy dual-phase alloys overcome the strength-ductility trade-off. Nature 534, 227–230 (2016).
-
Miracle, D. B. & Senkov, O. N. A critical review of high entropy alloys and related concepts. Acta Mater. 122, 448–511 (2017).
-
Yang, T. et al. Multicomponent intermetallic nanoparticles and superb mechanical behaviors of complex alloys. Science 362, 933–937 (2018).
-
George, E. P., Raabe, D. & Ritchie, R. O. High-entropy alloys. Nat. Rev. Mater. 4, 515–534 (2019).
-
Ren, J. et al. Strong yet ductile nanolamellar high-entropy alloys by additive manufacturing. Nature 608, 62–68 (2022).
-
Xie, P. et al. Highly efficient decomposition of ammonia using high-entropy alloy catalysts. Nat. Commun. 10, 4011 (2019).
-
Batchelor, T. A. A. et al. High-entropy alloys as a discovery platform for electrocatalysis. Joule 3, 834–845 (2019).
-
Xin, Y. et al. High-entropy alloys as a platform for catalysis: progress, challenges, and opportunities. ACS Catal. 10, 11280–11306 (2020).
-
Löffler, T., Ludwig, A., Rossmeisl, J. & Schuhmann, W. What makes high‐entropy alloys exceptional electrocatalysts? Angew. Chem. Int. Ed. 60, 26894–26903 (2021).
-
Sun, Y. & Dai, S. High-entropy materials for catalysis: a new frontier. Sci. Adv. 7, eabg1600 (2021).
-
Yao, Y. et al. High-entropy nanoparticles: synthesis-structure-property relationships and data-driven discovery. Science 376, eabn3103 (2022).
-
Koželj, P. et al. Discovery of a superconducting high-entropy alloy. Phys. Rev. Lett. 113, 107001 (2014).
-
Sarkar, A. et al. High entropy oxides for reversible energy storage. Nat. Commun. 9, 3400 (2018).
-
Li, W., Liu, P. & Liaw, P. K. Microstructures and properties of high-entropy alloy films and coatings: a review. Mater. Res. Lett. 6, 199–229 (2018).
-
Jiang, B. et al. High figure-of-merit and power generation in high-entropy GeTe-based thermoelectrics. Science 377, 208–213 (2022).
-
Tsai, M.-H. & Yeh, J.-W. High-entropy alloys: a critical review. Mater. Res. Lett. 2, 107–123 (2014).
-
He, Q. & Yang, Y. On lattice distortion in high entropy alloys. Front. Mater. 5, 42 (2018).
-
Zou, Y., Maiti, S., Steurer, W. & Spolenak, R. Size-dependent plasticity in an Nb25Mo25Ta25W25 refractory high-entropy alloy. Acta Mater. 65, 85–97 (2014).
-
Owen, L. R. et al. An assessment of the lattice strain in the CrMnFeCoNi high-entropy alloy. Acta Mater. 122, 11–18 (2017).
-
Song, H. et al. Local lattice distortion in high-entropy alloys. Phys. Rev. Mater. 1, 023404 (2017).
-
Lee, C. et al. Lattice distortion in a strong and ductile refractory high-entropy alloy. Acta Mater. 160, 158–172 (2018).
-
Li, J. et al. Heterogeneous lattice strain strengthening in severely distorted crystalline solids. Proc. Natl Acad. Sci. USA 119, e2200607119 (2022).
-
Chen, B. et al. Correlating dislocation mobility with local lattice distortion in refractory multi-principal element alloys. Scr. Mater. 222, 115048 (2023).
-
Zhang, F. X. et al. Local Structure and Short-Range Order in a NiCoCr Solid Solution Alloy. Phys. Rev. Lett. 118, 205501 (2017).
-
Ding, J., Yu, Q., Asta, M. & Ritchie, R. O. Tunable stacking fault energies by tailoring local chemical order in CrCoNi medium-entropy alloys. Proc. Natl Acad. Sci. USA 115, 8919–8924 (2018).
-
Ma, Y. et al. Chemical short-range orders and the induced structural transition in high-entropy alloys. Scr. Mater. 144, 64–68 (2018).
-
Li, Q. J., Sheng, H. & Ma, E. Strengthening in multi-principal element alloys with local-chemical-order roughened dislocation pathways. Nat. Commun. 10, 3563 (2019).
-
Ding, Q. et al. Tuning element distribution, structure and properties by composition in high-entropy alloys. Nature 574, 223–227 (2019).
-
Zhang, R., Chen, Y., Fang, Y. & Yu, Q. Characterization of chemical local ordering and heterogeneity in high-entropy alloys. MRS Bull. 47, 186–193 (2022).
-
Zhang, R. et al. Short-range order and its impact on the CrCoNi medium-entropy alloy. Nature 581, 283–287 (2020).
-
Chen, X. et al. Direct observation of chemical short-range order in a medium-entropy alloy. Nature 592, 712–716 (2021).
-
Walsh, F., Zhang, M., Ritchie, R. O., Minor, A. M. & Asta, M. Extra electron reflections in concentrated alloys do not necessitate short-range order. Nat. Mater. 22, 926–929 (2023).
-
Miao, J., Ercius, P. & Billinge, S. J. L. Atomic electron tomography: 3D structures without crystals. Science 353, aaf2157 (2016).
-
Ritchie, R. O. The conflicts between strength and toughness. Nat. Mater. 10, 817–822 (2011).
-
Gludovatz, B. et al. Exceptional damage-tolerance of a medium-entropy alloy CrCoNi at cryogenic temperatures. Nat. Commun. 7, 10602 (2016).
-
Zhang, Z. et al. Dislocation mechanisms and 3D twin architectures generate exceptional strength-ductility-toughness combination in CrCoNi medium-entropy alloy. Nat. Commun. 8, 14390 (2017).
-
Ma, E. & Wu, X. Tailoring heterogeneities in high-entropy alloys to promote strength–ductility synergy. Nat. Commun. 10, 5623 (2019).
-
Varvenne, C., Luque, A. & Curtin, W. A. Theory of strengthening in fcc high entropy alloys. Acta Mater. 118, 164–176 (2016).
-
Lu, K., Lu, L. & Suresh, S. Strengthening materials by engineering coherent internal boundaries at the nanoscale. Science 324, 349–352 (2009).
-
Otto, F. et al. The influences of temperature and microstructure on the tensile properties of a CoCrFeMnNi high-entropy alloy. Acta Mater. 61, 5743–5755 (2013).
-
Pedersen, J. K., Batchelor, T. A. A., Bagger, A. & Rossmeisl, J. High-entropy alloys as catalysts for the CO2 and CO reduction reactions. ACS Catal. 10, 2169–2176 (2020).
-
Nellaiappan, S. et al. High-entropy alloys as catalysts for the CO2 and CO reduction reactions: experimental realization. ACS Catal. 10, 3658–3663 (2020).
-
Pedersen, J. K. et al. Bayesian optimization of high-entropy alloy compositions for electrocatalytic oxygen reduction. Angew. Chem. Int. Ed. 60, 24144–24152 (2021).
-
Xie, S. et al. Atomic layer-by-layer deposition of Pt on Pd nanocubes for catalysts with enhanced activity and durability toward oxygen reduction. Nano Lett. 14, 3570–3576 (2014).
-
Cruz-Martínez, H. et al. NiPdPt trimetallic nanoparticles as efficient electrocatalysts towards the oxygen reduction reaction. Int. J. Hydrogen Energy 44, 12463–12469 (2019).
-
Wu, D. et al. Noble-metal high-entropy-alloy nanoparticles: atomic-level insight into the electronic structure. J. Am. Chem. Soc. 144, 3365–3369 (2022).
-
Yao, Y. et al. Carbothermal shock synthesis of high-entropy-alloy nanoparticles. Science 359, 1489–1494 (2018).
-
Xu, R. et al. Three-dimensional coordinates of individual atoms in materials revealed by electron tomography. Nat. Mater. 14, 1099–1103 (2015).
-
Chen, C.-C. et al. Three-dimensional imaging of dislocations in a nanoparticle at atomic resolution. Nature 496, 74–77 (2013).
-
Johnson, C. L. J. et al. Effects of elastic anisotropy on strain distributions in decahedral gold nanoparticles. Nature Mater. 7, 120–124 (2008).
-
De Fontaine, D. The number of independent pair-correlation functions in multicomponent systems. J. Appl. Crystallogr. 4, 15–19 (1971).
-
Li, T. et al. Denary oxide nanoparticles as highly stable catalysts for methane combustion. Nat. Catal. 4, 62–70 (2021).
-
Tian, X. et al. Correlating the three-dimensional atomic defects and electronic properties of two-dimensional transition metal dichalcogenides. Nat. Mater. 19, 867–873 (2020).
-
Yang, Y. et al. Atomic-scale identification of the active sites of nanocatalysts. Preprint at https://arxiv.org/abs/2202.09460 (2023).
-
Scott, M. C. et al. Electron tomography at 2.4-ångström resolution. Nature 483, 444–447 (2012).
-
Dabov, K., Foi, A., Katkovnik, V. & Egiazarian, K. Image denoising by sparse 3-D transform-domain collaborative filtering. IEEE Trans. Image Process. 16, 2080–2095 (2007).
-
Yang, Y. et al. Determining the three-dimensional atomic structure of an amorphous solid. Nature 592, 60–64 (2021).
-
Yuan, Y. et al. Three-dimensional atomic packing in amorphous solids with liquid-like structure. Nat. Mater. 21, 95–102 (2022).
-
Pham, M., Yuan, Y., Rana, A., Osher, S. & Miao, J. Accurate real space iterative reconstruction (RESIRE) algorithm for tomography. Sci. Rep. 13, 5624 (2023).
-
Lloyd, S. Least squares quantization in PCM. IEEE Trans. Inf. Theory 28, 129–137 (1982).
-
Yang, Y. et al. Deciphering chemical order/disorder and material properties at the single-atom level. Nature 542, 75–79 (2017).
-
Brünger, A. T. et al. Crystallography & NMR system: a new software suite for macromolecular structure determination. Acta Crystallogr. D 54, 905–921 (1998).
-
Zhou, J. et al. Observing crystal nucleation in four dimensions using atomic electron tomography. Nature 570, 500–503 (2019).
-
Pelz, P. M. et al. Simultaneous successive twinning captured by atomic electron tomography. ACS Nano 16, 588–596 (2022).
-
Stein, O., Jacobson, A., Wardetzky, M. & Grinspun, E. A smoothness energy without boundary distortion for curved surfaces. ACM Trans. Graph. 39, 18 (2020).
-
Zunger, A., Wei, S., Ferreira, L. G. & Bernard, J. E. Special quasirandom structures. Phys. Rev. Lett. 65, 353–356 (1990).
-
Krexner, G. & Hafner, J. Ab initio molecular-dynamics simulation of the liquid-metal–amorphous-semiconductor transition in germanium. Phys. Rev. B 49, 14251–14269 (1994).
-
Monkhorst, H. J. & Pack, J. D. Special points for Brillouin-zone integrations. Phys. Rev. B 13, 5188–5192 (1976).
-
Kresse, G. & Joubert, D. From ultrasoft pseudopotentials to the projector augmented-wave method. Phys. Rev. B 59, 1758–1775 (1999).
-
Perdew, J. P., Burke, K. & Ernzerhof, M. Generalized gradient approximation made simple. Phys. Rev. Lett. 77, 3865–3868 (1996).
-
Plimpton, S. Fast parallel algorithms for short-range molecular dynamics. J. Comput. Phys. 117, 1–19 (1995).
-
Zhou, X. W., Johnson, R. A. & Wadley, H. N. G. Misfit-energy-increasing dislocations in vapor-deposited CoFe/NiFe multilayers. Phys. Rev. B 69, 144113 (2004).
Acknowledgements
This work was supported by the US Department of Energy, Office of Science, Basic Energy Sciences, Division of Materials Sciences and Engineering under award no. DE-SC0010378. The ADF-STEM imaging with TEAM 0.5 was performed at the Molecular Foundry, which is supported by the Office of Science, Office of Basic Energy Sciences of the US DOE under contract no. DE-AC02-05CH11231. J.D. was supported by the Natural Science Foundation of China (grant no. 12004294) and the HPC platform of Xi’an Jiaotong University.
Author information
Authors and Affiliations
Contributions
J.M. initiated and directed the project; Y. Yao and L.H. synthesized the samples; J.Z., P.E. and J.M. discussed and/or carried out the AET experiments; Y. Yang, Y. Yuan, S.M. and J.M. performed image reconstruction, atom tracing and classification for the AET experiments. S.M., Y. Yang, Y. Yuan, L.Y., F.Z., Y.L., J.D. and J.M. analysed the data and interpreted the results; J.D. performed the DFT calculations and molecular dynamics simulations with input from S.M., Y. Yang, Y. Yuan and J.M.; J.M., S.M. and Y. Yang wrote the paper. All authors commented on the paper.
Corresponding author
Ethics declarations
Competing interests
The authors declare no competing interests.
Peer review
Peer review information
Nature thanks Paul Voyles and the other, anonymous, reviewer(s) for their contribution to the peer review of this work. Peer reviewer reports are available.
Additional information
Publisher’s note Springer Nature remains neutral with regard to jurisdictional claims in published maps and institutional affiliations.
Extended data figures and tables
Extended Data Fig. 1 EDS maps of the HEA nanoparticles.
a, Low-resolution ADF-STEM image of the nanoparticles. Scale bars, 20 nm. b-i, EDS maps showing the distribution of Co (b), Ni (c), Ru (d), Rh (e), Pd (f), Ag (g), Ir (h) and Pt (i) in the nanoparticles.
Extended Data Fig. 2 3D precision estimation.
a, b, Comparison between a representative experimental (after denoising) (a) and a multi-slice calculated image (b) of MEA-3. The multi-slice images were convolved with a Gaussian function to match the incoherence effects in the experimental images. Scale bar, 2 nm. c, Histogram of the deviation of the 3D atomic positions between the experimental atomic model and that obtained from 55 multi-slice images. The root-mean-square deviation of the histogram is 19.5 pm.
Extended Data Fig. 3 Experimental 3D atomic models of the other six M/HEA nanoparticles.
a-f, Experimental atomic models of four MEAs and two HEAs, named MEA-3 (a), MEA-4 (b), MEA-5 (c), MEA-6 (d), HEA-3 (e) and HEA-4 (f), in which the yellow circles represent the atoms along the twin boundaries in (a-c) and grey circles indicate the atoms on the grain boundary in (c). Scale bar, 1 nm.
Extended Data Fig. 4 Twin boundaries and dislocations in the M/HEAs.
a-e, The twin boundaries of three representative MEAs (a, twin-free; b, single twin; c, double twins) and two HEAs (d, single twin; e, double twins), showing more diffuse twin boundaries in the HEAs with each boundary spreading to the neighbouring atomic layers. The twin order parameter of 1 and −1 represents a hcp (i.e., twinning) and fcc structure, respectively. f, Two Shockley partial dislocations in MEA-5 with opposite Burgers vectors \(\frac{a}{6}\left[121\right]\) and \(\frac{a}{6}[\bar{1}\,\bar{2}\bar{1}]\), as the gliding process was frozen near the boundary during the rapid cooling process of the nanoparticle. g, A screw dislocation in MEA-2 with the Burgers vector \(\frac{a}{2}[110]\), where the zigzag lines in light blue show the characteristic feature of the screw dislocation. h, A Shockley partial dislocation in HEA-4 with the Burgers vector \(\frac{a}{6}\left[121\right]\), which exists near the boundary of the nanoparticle. i, A screw dislocation in HEA-3 with the Burgers vector \(\frac{a}{2}[110]\). Scale bars, 1 nm (a); and 5 Å (f).
Extended Data Fig. 5 3D distribution of the other four CSRO parameters of twin-free MEA-1 and double-twinned MEA-2.
a-d, 3D distribution of \({\alpha }_}\), \({\alpha }_}\), \({\alpha }_}\), and \({\alpha }_}\) in MEA-1, showing the formation of local chemical-order pockets. e-h, Histogram of the average \({\alpha }_}\), \({\alpha }_}\), \({\alpha }_}\), and \({\alpha }_}\) values for each atomic layer along the [111] direction in MEA-1. i-l, 3D distribution of \({\alpha }_}\), \({\alpha }_}\), \({\alpha }_}\), and \({\alpha }_}\) in MEA-2 (the twins labelled as yellow planes), exhibiting more heterogeneous CSRO than twin-free MEA-1 (a-d). m-p, Histogram of the average \({\alpha }_}\), \({\alpha }_}\), \({\alpha }_}\), and \({\alpha }_}\) values for each atomic layer along the [111] direction in MEA-2. Scale bar, 1 nm.
Extended Data Fig. 6 3D distribution of the six CSRO parameters in double-twinned MEA-3.
a-f, 3D distribution of \({\alpha }_}\), \({\alpha }_}\), \({\alpha }_}\), \({\alpha }_}\), \({\alpha }_}\), and \({\alpha }_}\), where the twins are labelled as yellow planes. g-l, Histogram of the average \({\alpha }_}\), \({\alpha }_}\), \({\alpha }_}\), \({\alpha }_}\), \({\alpha }_}\), and \({\alpha }_}\) values for each atomic layer along the [111] direction, where the yellow bars indicate the twin positions. Scale bar, 1 nm.
Extended Data Fig. 7 Twin formation energy (ETF) calculated from experimental 3D atomic coordinates of double-twinned MEA-3.
a-c, Calculation of ETF of the double-twinned MEA by fixing one twin (top yellow circles) and moving the other twin along the [111] direction, in which the three representative atomic configurations show a twin separation by 0 (i.e., a single twin) (a), 9 (b), and 13 atomic layers (c). d, Histogram of ETF as a function of the twin separation. The experimentally determined twin separation is 9 atomic layers (yellow bar), which is next to the minimum ETF with a twin separation of 10 layers. Scale bar, 1 nm.
Extended Data Fig. 8 3D distribution of the six CSRO parameters in twin-free HEA-1.
a-f, 3D distribution of \({\alpha }_{11}\), \({\alpha }_{22}\), \({\alpha }_{33}\), \({\alpha }_{12}\), \({\alpha }_{13}\), and \({\alpha }_{23}\), which are more heterogeneous than those of the twin-free MEAs (Fig. 3a,b, Extended Data Fig. 5a–d). g-l, Histograms of the average \({\alpha }_{11}\), \({\alpha }_{22}\), \({\alpha }_{33}\), \({\alpha }_{12}\), \({\alpha }_{13}\), and \({\alpha }_{23}\) values for the atomic layer along the [111] direction. Scale bar, 1 nm.
Extended Data Fig. 9 3D distribution of the six CSRO parameters in double-twinned HEA-2.
a-f, 3D distribution of \({\alpha }_{11}\), \({\alpha }_{22}\), \({\alpha }_{33}\), \({\alpha }_{12}\), \({\alpha }_{13}\), and \({\alpha }_{23}\), exhibiting greater local chemical fluctuations than the double-twinned MEA (Fig. 3e,f, Extended Data Figs. 5i–l and 6a–f). g-l, Histograms of the average \({\alpha }_{11}\), \({\alpha }_{22}\), \({\alpha }_{33}\), \({\alpha }_{12}\), \({\alpha }_{13}\), and \({\alpha }_{23}\) values of the atomic layer along the [111] direction. m, Histogram of the average values for the six CSRO parameters of a DFT-calculated bulk HEA, twin-free HEA-1, and double-twinned HEA-2. Scale bar, 1 nm.
Supplementary information
Rights and permissions
Springer Nature or its licensor (e.g. a society or other partner) holds exclusive rights to this article under a publishing agreement with the author(s) or other rightsholder(s); author self-archiving of the accepted manuscript version of this article is solely governed by the terms of such publishing agreement and applicable law.
About this article
Cite this article
Moniri, S., Yang, Y., Ding, J. et al. Three-dimensional atomic structure and local chemical order of medium- and high-entropy nanoalloys. Nature 624, 564–569 (2023). https://ift.tt/C4zAiJB
-
Received:
-
Accepted:
-
Published:
-
Issue Date:
-
DOI: https://ift.tt/C4zAiJB
Comments
By submitting a comment you agree to abide by our Terms and Community Guidelines. If you find something abusive or that does not comply with our terms or guidelines please flag it as inappropriate.
Bagikan Berita Ini
0 Response to "Three-dimensional atomic structure and local chemical order of medium- and high-entropy nanoalloys - Nature.com"
Post a Comment